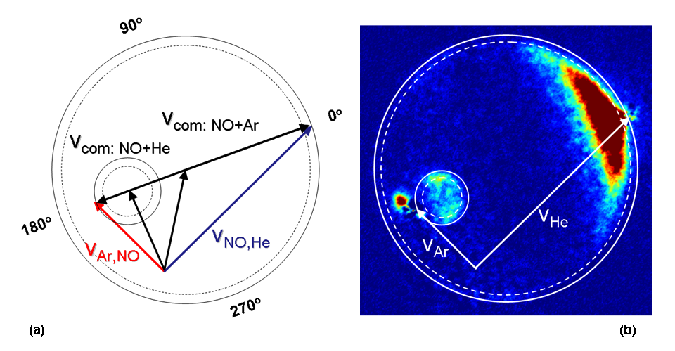
Collisional Energy Transfer Studied Under Single Collision Conditions
Ion imaging allows one to measure the differential cross section of a single quantum state of a collision process. The process could be either inelastic collisional energy transfer or reactive collisions. These studies are very sensitive tests of scientists’ ability to calculate the potential energy function between molecules and their ability to calculate dynamics upon that potential energy surface.
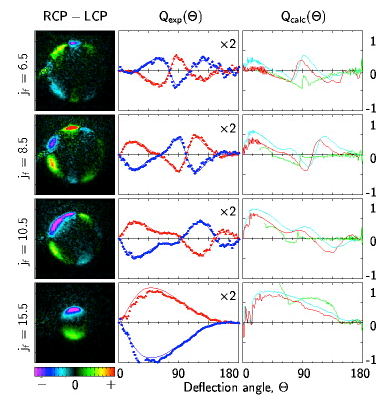
Initial experiments of inelastic collision were of ground state molecules having collision with atoms (NO, CO, H2, D2, HCL with He, Ne, Ar and Kr). These studies validated intermolecular potential energy surfaces and provided important information for modeling of combustion kinetics. Studies utilize laser ionization of a specific quantum state of a molecule and the projection of those ionized molecules onto an imaging detector to determine the velocity and direction of the scattering. Because a laser is used for the ionization, one can use the polarization properties of the laser to learn more information about the collision. In particular, one can learn how the angular momentum vector of product molecule is aligned in space (is the molecule spinning like a frisbee, a cartwheel or a propeller) as well as the orientation of the angular momentum vector (is the molecule rotating clockwise or counterclockwise with respect to the velocity vector of the molecule). The data set below was the first measurement of the orientation of the angular momentum vector of a product of a reaction and it was found to vary with the angle of scattering and the rotational state of the scattered product.
Images of specific quantum states of NO (jf) product after scattering NO(j=.5) from Argon and collecting the images with circularly polarized light. The patterns indicate that the product molecules in specific quantum states scattering at specific angles have a propensity to rotate either clockwise or counterclockwise and this propensity alternates more quickly with low rotational state products than with high rotational state products.
These ground state collisional studies also led to a new way to cool molecules to a few millikelvin temperature, best demonstrated by collision of Kr atoms form Kr atoms. When two molecular beams are crossed at 90o and the two atoms (or molecules) that collide weigh the same, then one of the possible scattering velocities that are available for elastic scattering that conserve both energy and angular momentum is zero. Think of playing a game of pool: the cue ball and the pool ball always scatter at 90o. If you were to run the pool shot backwards, you would see that scattering at 90 degrees can lead to one stationary ball (cold) and one moving ball (hot). The spot at the bottom of the image below on the left is of millikelvin temperature Kr atoms.
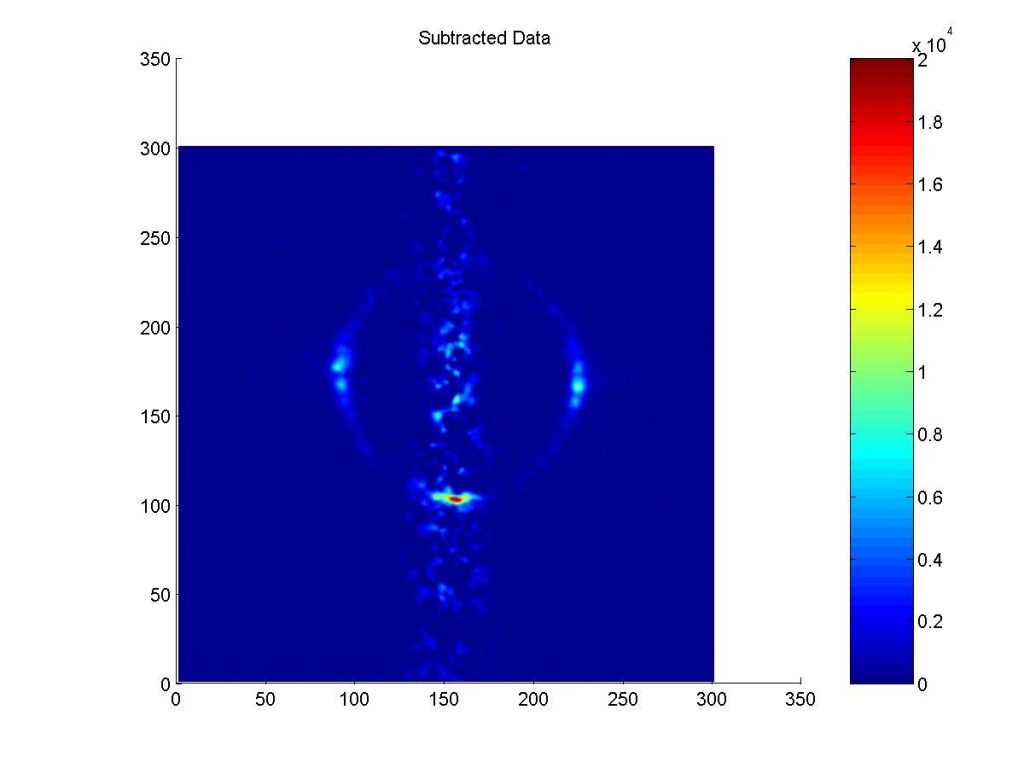
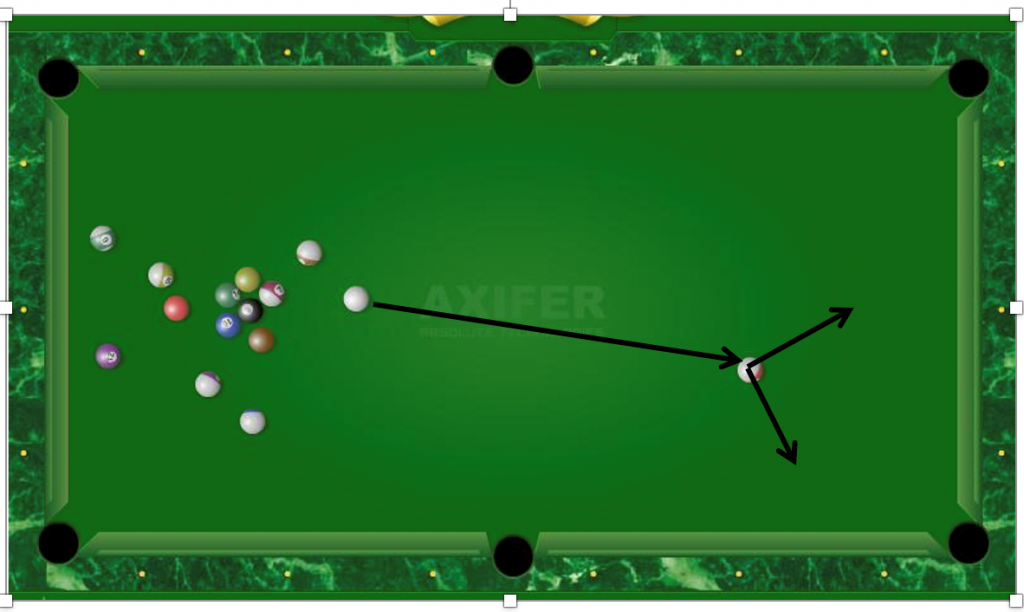
More recently we have pre-excited the molecule to be collided with to measure collisional energy transfer of electronically excited state molecule or highly vibrationally excited state molecules.
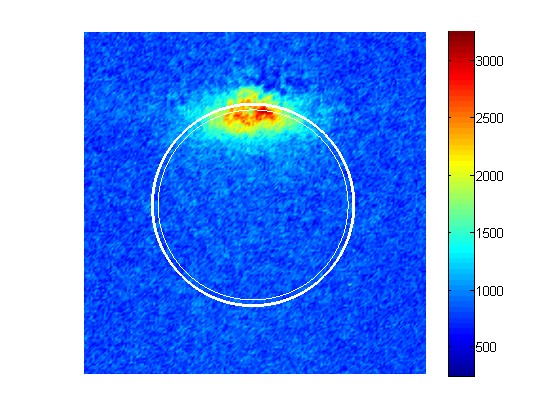
By electronically exciting NO2 just below the energy at which it dissociates the energy initially deposited into the electron is transferred into the ground electronic state and converts the energy into vibrational energy. A single collision with an Ar atom is then used to deposit enough energy into the vibrationally excited state molecule to dissociate the molecule. The NO (j) that is produced by the collision is then imaged onto a detector and the translational distribution measured. By measuring the velocity distribution of all the energetically possible quantum state one can reconstruct the collisional energy transfer function of the vibrationally excited state molecule.
By electronically exciting the NO molecule to the A state at the crossing of a molecular beam on and atomic beam on, we were able to measure the differential cross section, alignment and orientation of product NO(A) state molecules. Even though the NO(A) state has only a 200 nsec lifetime sufficient collisions occurred between the excitation laser and the detection laser (400 nsec) to record the data. Below is a sample of the data for different laser polarizations used to determine the orientation of the NO after the collision.
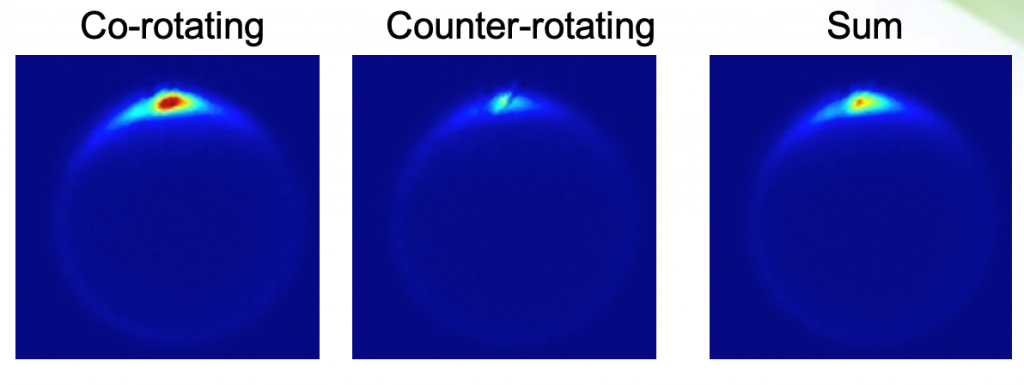
Future studies will utilize high resolution infrared lasers to excite a very narrow velocity distribution of the molecular beam and we will study extremely high velocity resolution scattering.
This demonstrates the capabilities in the laboratory to measure transient processes with unprecedented detail. We have not only measured this sort of differential cross section data we have also aligned the NO(A) to the direction of the collision and compared collisions of aligned NO molecules where the angular momentum vector is aligned parallel or perpendicular to the direction of the collision. This work is being done in collaboration with researchers Matt Costen and Ken McKendrick, both of Heriot Watt University in Scotland.
We utilize a crossed molecular beam machine with velocity mapped ion imaging ion optics to make the measurements.
Partners:
A partial list of recent collaborators includes Dr. David Parker, University of Nijmegen; Dr. James Valentini, Columbia; Dr. Joe Cline, University of Nevada, Las Vegas; Dr. Steven Stolte, Univ. of Amsterdam; Dr. Paul Houston, Cornell; Dr. Ahren Jasper, Argonne; Drs. Thomas Sharples, Mathew Costen, and Kenneth McKendrick, Harriot Watt University, Scotland; and Dr. Javier Aoiz, Madrid.
PI: David W. Chandler